In an article published last week in Nature Communications [1], Andrew Magyar and coworkers described a method for getting europium with a particular electronic structure into diamond. Before we examine the
how let's look for a second at the
why, as in, "why would you want to put europium into nanodiamond?"
Europium is an atom of a class called lanthanides, which have some pretty amazing properties.

|
Here they are! The Lanthanides are all in this row of the periodic table, named for the first in the series, lanthanum.
http://chemistry.tutorcircle.com/inorganic-chemistry/lanthanides.htm |
Lanthanides form colored compounds, and have very long lived luminescence, making them useful in all kinds of applications, from lighting, like in fluorescent bulbs, to tagging of materials and molecules to see how they move and change at a very small scale, to future applications including quantum computing.
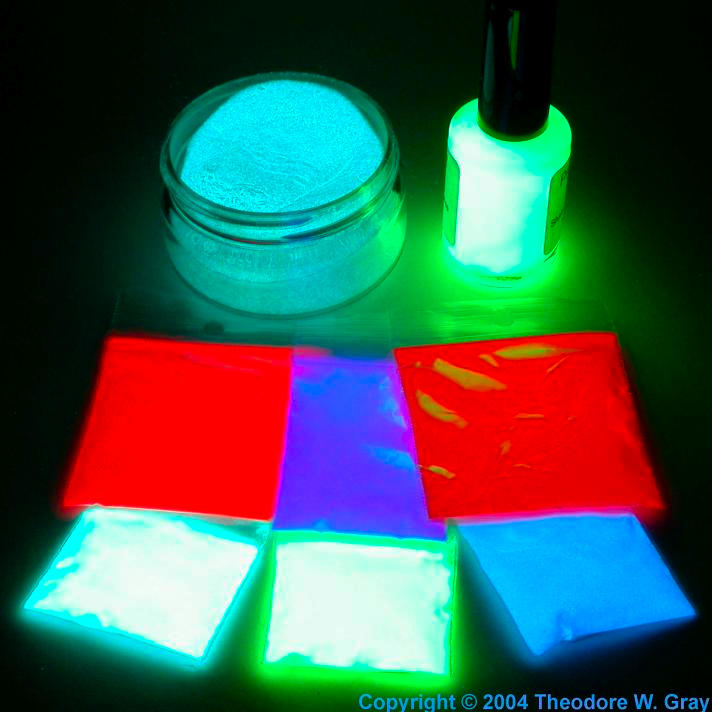 |
It glows! The green and aqua contain europium, and are the brightest available phosphorescent powders
http://www.periodictable.com/Items/063.7/index.html |
And what about nanodiamonds? Nanodiamonds are very small crystals of diamond, only several nanometers in size. A huge advantage for nanodiamond is that it can be functionalized, that is modified by adding things to the surface, in a way that other forms of diamond (like bigger crystals) often can't. They are also very small, and biocompatible. They can get into cells, and deliver drugs, like for cancer treatment, in a well controlled way. They can also be used as the seeds for the growth of continuous films of diamond. These films are called ultrananocrystalline diamond (UNCD) or nanocrystalline diamond (NCD) (it depends on how big the crystals of diamond are when they grow together), and they have lots of potential applications [3].
 |
A nanodiamond - This figure, from [2], shows the structure of a single nanodiamond particle |
So back to the original question, why do we want to get these lanthanides into these diamond particles? Diamond is seen as the material of the future for quantum computing, it has a couple of interesting defects, particularly the NV center, which I discussed briefly
in my last post. Incorporating europium into diamond could open up exciting applications for quantum memory, and by incorporating the luminescent europium into nanodiamonds, they can be used as biomarkers, allowing researchers to track things that are happening inside cells.
The problem is that it has been hard to get these atoms into the diamond and having them stay active the way they should in order to preserve these luminescence properties. Magyar mentions that in some previous work they tried ion implantation, without success. Ion implantation is the process of bombarding a surface, in this case a bunch of nanodiamonds, with very high energy atoms, for example europium atoms, and then hoping they will land inside the material, without too much damage to material. For most materials you can repair some of the damage you cause in the process by heating it afterward, but as I mentioned briefly
before, diamond doesn't heal unless you put it under a lot of pressure while you heat it. As a result, getting europium atoms into the nanodiamonds was a bit tricky, and so required a trick. And that trick is... (cue epic music) electrostatic assembly.
Electrostatic assembly is by no means a new trick, it is the method, for example, that printers and copiers use to position toner on paper. There isn't just one way to do it either, a number of different sources of the charging, from large electrodes down to the tip of a nanometer sized probe can be used to guide the assembly. Proving that europium can be put into diamond this way could mean that researchers may now be able to effectively "print" not just europium, but a wide variety of atoms onto a growth surface, and incorporate them into grown diamond films.
The trick here is that they can't just put the europium on the diamond surface and then grow and get the desired results. Europium forms oxides (bonds with oxygen) easily, and the oxides make it really unlikely that the europium will incorporate itself into the diamond when they grow on it. To do the electrostatic assembly, it's necessary to have an oxidized surface, since the oxygen acts as the negative charge of the electrostatic attraction. To keep the europium safe from the oxidized surface, Magyar and coworkers used a polymer (polymer is essentially a fancy way of saying plastic) as a layer between the europium and the oxidized surface.
They found the best results when they formed an oxidized surface on a wafer of silicon dioxide (it's a common substrate, and really easily formed in modern silicon fabrication processes like what are used to make computer chips). This is shown in the figure below where the blue circles with the negative charges in them are oxygen. Just to be clear, in the figure below they seem to be saying that the surface is diamond, but in their most successful method it was actually not, it was silicon dioxide. (The nanodiamonds will be added in the last step). So, after they oxidized the silicon dioxide, they used electrostatic assembly to put down the polymer layer on top. Next comes a layer of europium, which is modified so that it is able to be used this way by surrounding it with carbon atoms (the pink dot in the figure below is europium, the grey material around it represents carbon atoms). They then spread nanodiamonds over the surface, and put it into a diamond growth reactor. I won't get too into the details of diamond growth in this post (but I definitely plan to in a later post), essentially they grew the diamond long enough that the individual nanodiamonds got larger, but stayed individual nanodiamonds without growing all together into a single film.
 |
The method for assembling europium on the surface before growth (from [1]) |
Magyar and coworkers also tried this method for chromate, a source of another well known luminescent defect in diamond, and were successful with the chromate as well. As a result, they believe it can be extended to a bunch of other atoms, essentially anything that can be electrostatically assembled.
So what does it all mean? In addition to luminescence related applications, like tagging of nanodiamonds for biological visualization, there are also other potentially interesting ways that patterning diamond with lanthanides and other interesting atoms could unlock new technologies. Patterning is always a useful technique for electronics, and having a way to localize interesting atoms with techniques engineered based on this method using electrostatic assembly could unlock a whole host of new, cool diamond electronic and (and optoelectronic!) devices.
References
[1]
Magyar, A., Hu, W., Shanley, T., Flatté, M., Hu, E., & Aharonovich, I. (2014). Synthesis of luminescent europium defects in diamond Nature Communications, 5 DOI: 10.1038/ncomms4523
[2]
Mochalin, V., Shenderova, O., Ho, D., & Gogotsi, Y. (2011). The properties and applications of nanodiamonds Nature Nanotechnology, 7 (1), 11-23 DOI: 10.1038/nnano.2011.209
[3]
Williams, O., Nesladek, M., Daenen, M., Michaelson, S., Hoffman, A., Osawa, E., Haenen, K., & Jackman, R. (2008). Growth, electronic properties and applications of nanodiamond Diamond and Related Materials, 17 (7-10), 1080-1088 DOI: 10.1016/j.diamond.2008.01.103